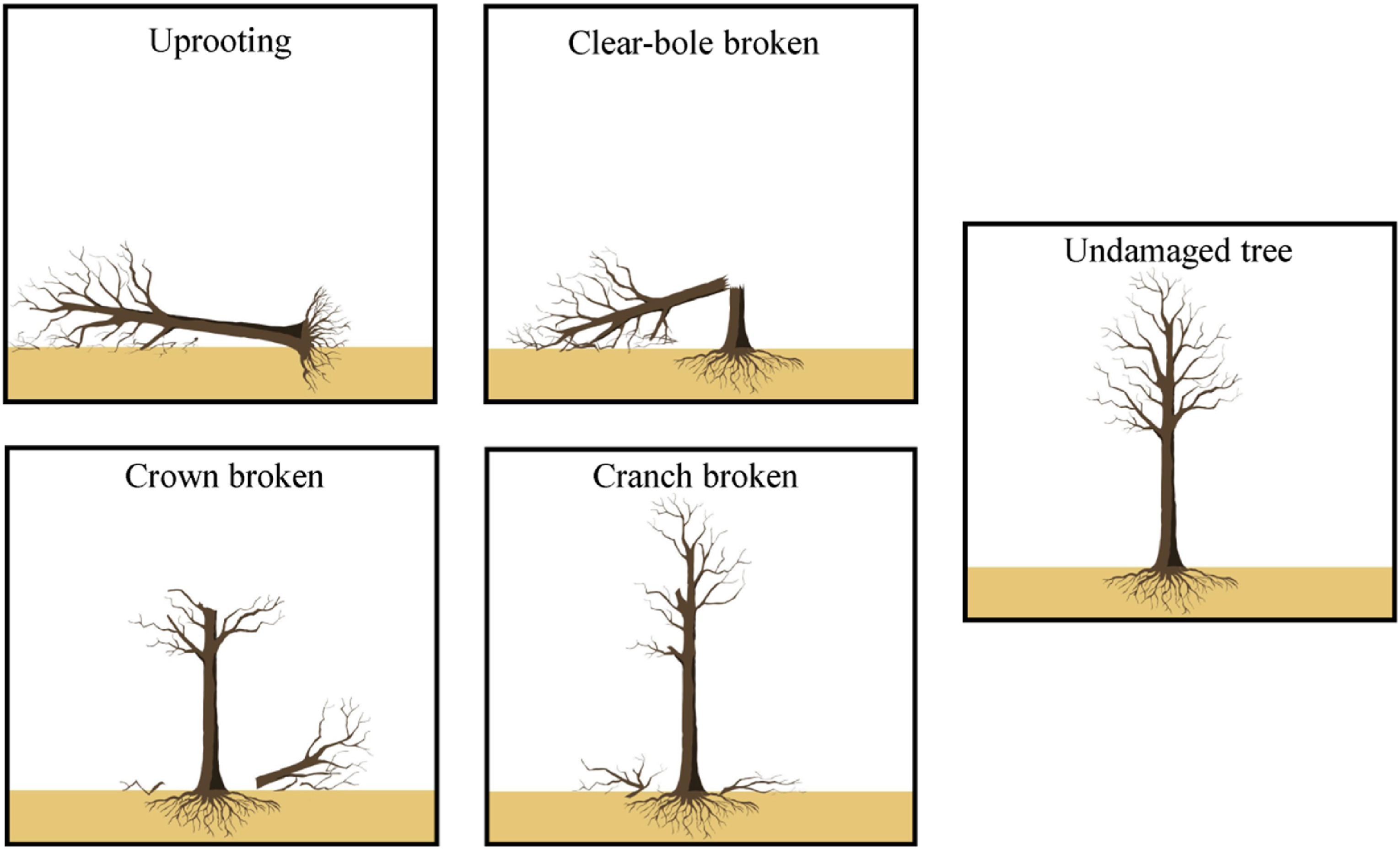
Citation: | Jinyu Guo, Jifa Cui, Nan Wu, Yaqian Zhang, Jie Wang, Hanyu Xiang, Baoshuang Hu, Youbing Zhou. Ice storm damage to oak forests in subtropical China[J]. Forest Ecosystems, 2023, 10(1): 100119. DOI: 10.1016/j.fecs.2023.100119 |
The purpose of this article is to report the effects of an ice storm on oak forests by comparing the damage in early, late and old-growth stands in subtropical China. More than 50% of the global forest cover is the secondary-growth stand and this proportion is expected to expand in the future due to human-induced pressures (Curtis et al., 2018; Wang et al., 2020; Estoque et al., 2022). Regrowth forests are becoming important for biodiversity conservation (Matos et al., 2020; Chen et al., 2020) and climate change mitigation (Bongers et al., 2015; Pugh et al., 2019; Anderson-Teixeira et al., 2021). Average carbon sequestration rates of secondary-growth forests can reach 3.05 Mg C·ha−1·yr−1, which is 20 times faster than the old-growth forests (Requena Suarez et al., 2019; Heinrich et al., 2021). Although these secondary-growth forests do not contain all the species in the regional species pool, they still harbor a high level of biodiversity (Rozendaal et al., 2019; He et al., 2022). Furthermore, secondary-growth forests emerging from natural regeneration can provide sustainable ecosystem services to local communities (e.g., forest product provisioning) and society (e.g., climate change mitigation) (Naime et al., 2020; Hua et al., 2022). Thus, secondary-growth forests can provide multiple benefits at global, national, regional and local levels (Pugh et al., 2019; Tong et al., 2020; Matos et al., 2020).
With global warming, climatic extremes such as heat waves, cold snaps, cyclones, droughts, and unusual rainfall are increasing in frequency, duration, and intensity (Diffenbaugh, 2020). Recently, the effects of extreme weathers on forest ecosystems have received increasing attention, because they induce episodes of extensive vegetation damage, consequently remolding forest carbon sinks and regulating subsequent carbon sequestration rates (Naime et al., 2020; Gnanamoorthy et al., 2021; Espunyes et al., 2022). These effects alter forest properties and mediate resilience dynamics in the short-term (Stone, 2008; Uriarte et al., 2019), and drive long-term hysteretic forest functioning trajectories including community shifts (Deschênes et al., 2019) and the reorganization of ecosystem structure, function and services (Zhou et al., 2013). Forest stands are linked to tree age and height, and have different responses to extreme climates. Several studies have shown that old-growth forests are susceptible to wind-related climatic extremes (e.g., hurricane, Kupfer et al., 2008; Ni et al., 2021; windstorm, Bouchard et al., 2009), whereas secondary-growth forests are vulnerable to droughts and wildfires (Lindenmayer et al., 2020, 2022; Bowman et al., 2021). Temperature extremes pose a risk to plant survival if they occur at the limits of the species' thermal niches (Lancaster and Humphreys, 2020; Girardin et al., 2022), particularly for pioneer tree species in secondary-growth forests (Benkov et al., 2019; Kunert and Hajek, 2022).
Ice storm (also called 'glaze'), as a common agent of frequent and injurious disturbances in the Northern Hemisphere temperate region, has been a focus of attention in recent decades (Deschênes et al., 2019; Roženbergar et al., 2020). Compared to the damage patterns caused by other extreme disturbance agents (e.g., frost, hurricane), ice storms typically result in severe damage (particularly uprooted or snapped trees) with a high proportion of crown loss over large regions (Bragg et al., 2003; Roženbergar et al., 2020), and alter the consequent forest regeneration dynamics, species interaction networks and soil biogeochemical cycles over the long term (Zhou et al., 2013, 2021; Deschênes et al., 2019). However, extreme ice storms, which have been rare in tropical and subtropical regions in the past, are predicted to become more frequent as a consequence of global warming and could be detrimental to subtropical forest ecosystems, particularly at high elevations (Gnanamoorthy et al., 2021; IPCC et al., 2021). Although previous studies have assessed the impact of ice storms on secondary- and old-growth forests, these assessments were conducted in isolation and focused on old-growth forests in the northern temperate zone (e.g., Rhoads et al., 2002; Roženbergar et al., 2020). Research on the differential impacts of ice storms on secondary- and old-growth forests remains limited, particularly in subtropical forests. The pattern of these different impacts is crucial for forest managers and landowners to take specific actions to limit the negative effects of ice storms (Bragg et al., 2003; Priebe et al., 2018).
Oaks (Quercus spp.), as climax and keystone species, dominate many forested land areas in the eastern deciduous forest biome (Shumway et al., 2001; McShea and Healy, 2002; Hanson et al., 2004). Oak forests are among the most productive ecosystems on the planet, providing a critically important habitat for numerous organisms, thereby harboring high biodiversity (McShea and Healy, 2002; Caleño-Ruíz et al., 2018; Rathore et al., 2019). Their multi-layered and dense canopies also play essential roles in CO2 capture, improving soil fertility, and regulating of the hydrological cycle by retaining soil moisture (Hanson et al., 2004, 2005; Xie et al., 2014; Poudel et al., 2020).
In this study, we examined the consequences of climatic perturbations on secondary- and old-growth forests and identified the potential determinants of tree damage at a World Natural Heritage Site in China. We used previously established long-term plots in oak forests to study plant-animal interactions and forest dynamics (Lei, 2021; Cui, 2022). In 20–21 March 2022, the study area experienced the worst ice storm recorded since 1975 (http://www.nmc.cn), which caused major damage to oak and oak-beech forests over 230,300 ha, according to immediate local government surveys using aerial photography and satellite imagery (http://lgj.snj.gov.cn/). Freezing rain contributed to ice accumulation over a 24-h period and up to 15 cm of ice formed on branches in the most affected areas. The ice generally melted within 48–72 h after the ice storm.
Our purpose was to investigate the occurrence, type and severity of ice storm damage in three oak forest stands (early, late and old-growth) by addressing the following questions.
ⅰ) Does the damage intensity caused by the 2022 ice storm differ among the three forest stands? Previous surveys of old-growth forests found that glaze damage intensity tended to show a non-linear inverted U-shaped curve with tree age (less damage to small and large individuals, but more damage to mid-sized trees; Rhoads et al., 2002; Priebe et al., 2018). Therefore, we predicted (P1) that late secondary-growth forests would be more sensitive to ice storms and would suffer greater damage than early secondary-growth and old-growth forests.
ⅱ) How did the severity of different damage types vary across the three forest stands? In general, ice storms result in more severe damage to small trees (e.g., uprooting) than to large ones (e.g., branch loss; Rhoads et al., 2002; Bragg et al., 2003; Karban and Pearse, 2021). Therefore, we predicted (P2) that the preponderant type of damage is 'branch broken' for the old-growth forest and 'uprooting' for the early secondary-growth forest, and there are similar damage intensities for all damage classes in the late secondary-growth forest.
ⅲ) If P1 and P2 hold true, how do abiotic and biotic factors account for these differences? Two broad groups of factors affect forest vulnerability to ice storms: (1) abiotic factors, including topographical and soil variables; and (2) biotic factors, including forest structure, composition and nutrient input (Bragg et al., 2003; Deschênes et al., 2019). For abiotic factors, topography as a key environmental filtering variable, tends to play a more important role in determining damage intensity in early secondary-growth forests than in late secondary-growth and old-age forests because early secondary-growth forests are dominated by early-successional species (Song et al., 2018). Soil variables influence the damage intensity by providing the anchorage role of soil-root architecture (Priebe et al., 2018; Taylor et al., 2019). For biotic factors, the damage of ice storms will link to forest structure and composition, but their roles in resisting damage intensity are complex (Bragg et al., 2003; Priebe et al., 2018; Taylor et al., 2019). Generally, trees with small, less dense crowns and high-density understory shrub layers suffer less glaze damage, whereas trees with strong stem wood and deep rooting habits are prone to branch breakage (Rhoads et al., 2002; Bragg et al., 2003; Karban and Pearse, 2021). Therefore, we predicted (P3) that, among the three stands, abiotic factors are the most important for early secondary-growth forests because they comprise low density vegetation dominated by pioneer species. Among the three stands, biotic factors are the most important for old-growth forests because their vegetation is complex and multi-layered. Both abiotic and biotic factors are associated with damage intensity in late secondary-growth forests.
Data were collected from the Shennongjia World Natural Heritage Site (31°19′ N, 110°29′ E; Fig. S1), Hubei Province, central China, which is a national and international biodiversity conservation hotspot. This region harbors exceptional biodiversity and is representative of the oriental deciduous forest biogeographical province (Chen et al., 2019; Xie and Shen, 2021). The study area lies in the transitional zone from the mid-subtropical belt to the north subtropical belt and is characterized by four distinct seasons, including a hot, humid summer (June–August, monthly mean temperature > 25 ℃, monthly mean precipitation > 250 mm), a cold winter (December–February, monthly mean temperature < ‒4 ℃; Chen et al., 2019), moderate autumn (September–November) and spring (March–May; Zhu, 2011). Annual precipitation ranges from 1,306 to 1,722 mm, and the annual temperature averages 10.6 ℃. Economic logging occurred in the Shennongjia region from 1953 until 1999 when the 'Grain-for-Green' environmental protection program was implemented during 1997–1999, which resulted in a large area of early and late secondary-growth forests (Zhu, 2011; Xie and Shen, 2021).
The set of permanent plots used in this study was established before the 2022 ice storm (Lei, 2021; Cui, 2022). These sites were used as part of a project to study the diversity and function of subtropical plant communities (Chen et al., 2019; Xie and Shen, 2021). Oaks forests were the main vegetation cover type in our plots. Sharptooth oak (Quercus aliena var. acuteserrata) is a dominant component of the canopy (60%–90% relative abundance), and adult sharptooth oak trees can reach heights of approximately 30 m (He, 1998; Zhu, 2011; Chen et al., 2019). A total of 108 plots (20 m × 20 m quadrats) representing three forest stands (early and late secondary-growth forest, and old-growth forest) were selected as they were similarly struck by the 2022 ice storm (http://lgj.snj.gov.cn/).
To evaluate the differential impacts of the 2022 ice storm on forest stands, sites are representative of three forest stands we classified based on tree size and historical records regarding the last logging (Requena Suarez et al., 2019).
Stand-1) Early secondary-growth forest (20–40 years): this forest type was dominated by small trees with a mean diameter at breast height (DBH, following Fang et al. (2009), Fig. S2) of 11.7 cm and a mean height (the tree height measured by visual estimation, Fig. S3) of 10.0 m after development following clear cut 20–40 years ago.
Stand-2) Late secondary-growth forest developed by natural succession from clear cut 40–60 years ago, with medium-sized trees (15.4 cm and 12.0 m in mean DBH and tree height).
Stand-3) Old-growth forest (more than 150 years old): this forest stand was characterized by a high level of complexity of vertical and horizontal structures with many large old trees. We carefully selected forest stands that had been protected as part of the forest tree provenance and were never logged (Xu, 1996; Wang et al., 2007).
During the two months after the 2022 ice storm, the post-storm damage caused to vegetation was assessed. At each of the three forest stands, six replicate transect sites were established in two areas (Fig. S1), approximately 300 m long and at least 500 m apart (Lei, 2021; Cui, 2022). Each transect site was subdivided into six monitoring plots (each at the center of a 20 m × 20 m square, 50-m distance parallel to the roadside). The plots were spaced at approximately 50 m apart. Thus, 108 plots were obtained (two sampling areas × three forest stands × three transect sites × six monitoring plots). The location of each transect site was randomly selected within each sampling area, and topographic factors were different for each transect site in the 3.99 km (Fig. S1a) and 2.64 km (Fig. S1b) ranges to avoid simple pseudo-replications (Hurlbert, 1984; Oksanen, 2001; Davies and Gray, 2015). All the plots have similar slopes (22.9° ± 1.48[SE]°, 19.5° ± 1.01° and 22.3° ± 1.64° for early and late secondary-growth forests, and old-growth forests, respectively; Fig. S4). The soil type at all transect sites was mountain brown forest soil.
In each plot, we surveyed and assigned individual trees to one of the five categories of damage previously commonly used (Bragg et al., 2003; Roženbergar et al., 2020). Plant leaves were absent when the 2022 ice storm occurred in March, which had limited effects on shrubs and herbs (Priebe et al., 2018; and our field observation). Specifically, we assigned individuals to one of the following categories: (1) uprooting, (2) clear-bole broken, (3) crown broken (i.e., stem broken above clear-bole), (4) branch broken, and (5) undamaged tree (Fig. 1).
Five groups of explanatory variables belonging to abiotic and biotic factors were selected based on our predicted influence on forest vulnerability to ice storms: (1) topography, (2) soil, (3) forest structure, (4) forest composition, and (5) nutrient input (Table 1). These variables have previously been found to affect the intensity of damage caused by ice storms (Bragg et al., 2003; Deschênes et al., 2019; Klopčič et al., 2020).
Factor | Early second-growth forest | Late second-growth forest | Old-growth forest | ||||||||||||||||
Category | Parameter | UP | CBB | CB | BB | TO | UP | CBB | CB | BB | TO | UP | CBB | CB | BB | TO | |||
Topography | Slope gradient | ↑*** | ↑*** | ↑*** | – | – | – | – | – | ↑*** | |||||||||
Slope aspect | ↑* | ↑** | ↑*** | ↓**** | ↓** | ↓** | ↓**** | – | – | – | – | – | |||||||
Soil | Soil hardness | ↓* | ↓** | ↓** | |||||||||||||||
Percent of bare rock | |||||||||||||||||||
Forest structure | Height of tree layer | ↑** | ↑*** | ↑* | ↑** | ↑*** | |||||||||||||
Basal area of Tree layer | – | – | – | – | – | – | – | – | – | – | ↓** | ↑* | |||||||
Height of shrub layer | – | – | – | – | – | ↑** | ↑*** | ↑*** | |||||||||||
Basal area of shrub layer | – | – | – | – | – | ↓* | ↓** | ↓** | ↓** | ||||||||||
Forest composition | Density of tree layer | – | – | – | – | – | ↓** | ↓*** | ↓**** | – | – | – | – | – | |||||
Density of shrub layer | – | – | – | – | – | – | – | – | – | – | |||||||||
Height ratio of shrub to tree | – | – | – | – | – | – | – | – | – | – | – | – | – | – | – | ||||
Basal area ratio of shrub to tree | – | – | – | – | – | – | – | – | – | – | – | – | – | – | – | ||||
Density ratio of shrub to tree | – | – | – | – | – | – | – | – | – | – | ↓* | ↓* | ↓** | ||||||
Nutrient input | Litter thickness | ↓** | ↓* | ↓*** | ↑* | ||||||||||||||
Note: "↑" indicates a positive coefficient, and "↓" indicates a negative coefficient. "‒" indicates a variable that was not included in the final most plausible model. Significance levels of the explanatory variables (see Table S3 for statistical values) are shown as: *, P < 0.1; **, P < 0.05; ***, P < 0.01; ****, P < 0.001. |
Indices of topography (slope gradient and aspect) and soil (soil hardness and percentage of bare rock) were measured at the plot level. The slope gradient was measured by hand-held compass, a base application of the smartphone (i.e., iPhone 11), and calculated as the mean of the measured values from the four corners and center of each 20 m × 20 m focal plot (Song et al., 2018). The slope aspect was measured using a GPS device (GPSMAP®63sc, Garmin) at the focal quadrat center and cosine-transformed to continuous values, ranging from 1 (south) to −1 (north). Soil hardness (kg·cm−2) were surveyed by a hand-held soil penetrometer (TYD-2, Wenzhou Jingfeng Instrument Co., Wenzhou, China), which was pressed slowly and penetrated 10 cm into the soil (Fig. S5; Xie et al., 2013). The percentage of bare rock, defined as the abundance and distribution of rock fragments > 25 cm and measured as the percentage of surface soil covered by stone within each 20 m × 20 m focal plot, is an indicator of soil surface stoniness referring to soils dominated by decomposing plant material rather than mineral substrates (Taylor et al., 2019).
Ten parameters of forest structure (height and basal area of tree and shrub layers), composition (density of tree and shrub layers, height, basal area and density ratios of shrubs to trees), and nutrient input (litter thickness) were surveyed within each 20 m × 20 m quadrat (Ni et al., 2021). Basal area and density were measured as the sum of all individuals, and height was calculated as individual values averaged from each 20 m × 20 m focal plot. Litter thickness, an important indicator of nutrient input and conservation in forest ecosystems, was surveyed using a multiple tine litter collector (a rapid and efficient protocol for measurement of the litter layer (Fig. S6); Marimon-Junior and Hay, 2008). These litter layers were from years previous to the ice-storm as this ice storm occurred in the non-growing season of deciduous oak forests (i.e., 20–21 March 2022, Fig. S7). Similar to the slope gradient and soil hardness, litter thickness was calculated as the mean of the measured values from the four corners and the center of each focal plot (Song et al., 2018).
To quantify the extent of damage caused by the ice storms, the damage rates were calculated as the number of damaged trees (height > 5 m) divided by the total number of trees (height > 5 m) over a 20 m × 20 m quadrat for each damage category and total damaged trees. Relative damage ratios, defined as the rate of each damage type divided by the total damage rate, were calculated to test our second prediction (P2). Six plots were excluded from statistical analyses because they were partly logged for power lines (three plots in old-growth forests) and Gastrodia elata (a traditional Chinese medicine) planting (three plots in early secondary-growth forests) prior to the 2022 ice storm. All damage metrics were recorded as proportional data and fitted to generalized linear mixed-effect models (GLMM) with binomial distributions, which is a useful tool to account for dependencies within hierarchical groups of data through the introduction of random effects (Zuur et al., 2009). We included plots nested in the transect sites as random factors in these models to reduce potential pseudo-replication effects (Hurlbert, 1984; Oksanen, 2001; Davies and Gray, 2015). The results should be interpreted considering this; however, given the extent of the treatment and sample size (i.e., 102 plots), the differences found are most likely treatment effects rather than differences in physical characteristic among forest stands. Forest stand was a fixed factor for models of the damage rate, and forest stand, damage category and their interaction were used as fixed factors in the model to analyze the relative damage ratio. When a statistically significant interaction was found, separate models were run for each forest stand to better understand the relationship structure (Zuur et al., 2009; Chen et al., 2019). Multiple comparisons among forest stands and damage categories were conducted using Tukey's HSD multiple comparison test for all models.
To answer our third question, similar GLMMs were employed, where abiotic and biotic factors were included as fixed factors and the plots nested in the transect sites were included as random factors. As the initial 14 explanatory variables belonging to five groups (see above) were selected, we first minimized multicollinearity using variance inflation factor (VIF) analysis with a maximum threshold of two (Zuur et al., 2009), using the function corvif (http://www.highstat.com/Book2/HighstatLibV6.R). Additionally, the Pearson's correlation coefficients among the variables were calculated to detect redundancy. If two explanatory variables had a correlation coefficient of > 0.6, the variable that was less correlated with the response variables was removed from further analysis. A P value of 0.05 is customarily used for the statistical significance test, however, in this study, following Clauset et al. (2009), conservative P values of 0.10 were used as marginal significances, that is, if the P value obtained from simulations is > 0.10, there is no reason to refuse the hypothesis that the MLE parameters produce a statistically significant model of the observed data, and we can accept the hypothesis that the observed values follow the type of distribution that is being tested (Patiño Douce, 2016). Ultimately, only optimal explanatory variables were used in the most plausible models. All statistical analyses were conducted using R version 4.0.2, glmmTMB, multcomp, and Hmisc packages.
In total, 5,526 trees were recorded across a tree layer of 102,400 m2 plots. Of the 1,168 (21.14%) damaged individuals, 146 (2.64%), 210 (3.80%), 535 (9.68%) and 277 (5.01%) belonged to the categories 'uprooting', 'clear-bole broken', 'crown broken', and 'branch broken', respectively. The damage rate varied among damage categories and forest types (Fig. 2; Table S1). The total proportion and number of damaged trees in late secondary-growth (30.47%, 494 individuals) and old-growth forests (27.34%, 407 individuals) were almost twice as high as those in early secondary-growth forests (13.41%, 267 individuals) (Fig. 2a). The most common category of damage across three forest types was 'crown broken', followed by 'branch broken', 'clear-bole broken' and 'uprooting' (Fig. 2b–e). Two severe types of damage ('clear-bole broken' and 'uprooting') had similar patterns and the proportions were (marginally) significantly higher in the late secondary-growth forest (10.68% in total) than in the early secondary-growth (4.38%) and old-growth forests (5.63%) (Fig. 2b and c; Table S1). In contrast, the patterns of the two less severe types of damage ('crown broken' and 'branch broken') were also similar, and damage rates were significantly higher in the late secondary-growth (19.80% in total) and old-growth forests (21.71%) than in the early secondary-growth forest (9.03%) (Fig. 2d and e; Table S1). These results show that trees in late secondary-growth forests were most affected by the ice storms, whereas they were less affected in early secondary-growth forests, completely supporting our first prediction.
The relative damage rates showed similar patterns to the proportion of damage; the relative rates of trees corresponding to the category 'crown broken' (> 40%) were the highest across the three forest types (Fig. 3; Table S2). The relative rates of trees corresponding to the category 'branch broken' were marginally higher than those of the two severe types of damage in the early (Fig. 3a) and late secondary-growth forests (Fig. 3b), whereas this metric was significantly higher in the old-growth forest (Fig. 3c).
In the early secondary-growth forest, damage rates were strongly correlated with topographic parameters (Tables 1 and S3). Proportions of trees corresponding to two severe damage categories and total damage rate significantly increased with slope gradient (P < 0.008 for all cases; Table S3) while the proportions of trees belonging to the categories 'clear-bole broken' and 'branch broken', and total damage rate were (marginally) significant correlations with slope aspect (P < 0.078; Table S3). In addition, the parameter of nutrient input (i.e., litter thickness) showed (indicative) significant negative relationships for the proportions of trees corresponding to the 'uprooting' and 'branch broken', and total damage rate categories (P < 0.053; Table S3).
In general, topography, forest structure and composition were important factors explaining the damage rates in late secondary-growth forests (Tables 1 and S3). Damage rates, except for the 'branch broken' category, were significantly correlated with slope gradient (P < 0.048; Table S3). The height and basal area of the shrub layer showed a significant positive and indicative negative relationship with the proportion of individuals corresponding to the 'crown broken' category (P = 0.012 and 0.062; Table S3), indicating that stems above the clear-bole were easier to break when shrubs were tall and slim. Tree layer height had an aggravating effect on total damage rate (P = 0.042; Table S3), while its density showed decreasing effects on damage rates (P < 0.05), except for the categories 'uprooting' and 'branch broken'. Moreover, the proportion of trees corresponding to the 'uprooting' category had an increasing trend with soil hardness (P = 0.061; Table S3).
Forest structure was the most important factor explaining the damage rates in old-growth forests (Tables 1 and S3). Tree layer height showed (marginally) significant aggravating effects on the damage rates (except for the category 'uprooting', P < 0.076 for all cases; Table S3) while its basal area had significant negative and indicative positive relationship with the categories 'clear-bole broken' (P = 0.042; Table S3) and 'branch broken' (P = 0.084). Similar to the late secondary-growth forests, trees in the old-growth forests were more easily damaged by ice storms when shrubs were tall and slim. The shrub to tree density ratio showed (marginal) significant decreasing effects on the damage rates (P < 0.076 for all cases; Table S3), except for the 'uprooting' and 'crown broken' categories. Meanwhile, the proportion of trees corresponding to the 'clear-bole broken' category significantly increased with slope gradient (P = 0.002; Table S3) while the proportion to 'crown broken' and total damage rate significantly declined with slope aspect (P = 0.012 and 0.062). The proportion of trees corresponding to the 'uprooting' category showed an increasing trend with litter thickness (P = 0.073; Table S3).
To date, there have been no empirical evaluations of how different forest stands respond to ice storms in the subtropics. Here, we assess the effects of a severe ice storm on three forest stands (early and late secondary-growth forests, and old-growth forest); and the determining role of abiotic and biotic factors on the damage intensity. The highest damage intensity in the late secondary-growth forest confirmed our first prediction. Although no difference was found in two severe types of damage (i.e., 'clear-bole broken' and 'uprooting'), high relative proportion of trees corresponding to two less severe types of damage (i.e., 'branch broken' and 'crown broken') in the old-growth forests revealed that the ice storm caused the crown loss for old-age trees, partly supporting our second prediction. Consistent with our third prediction, abiotic factors were highly related to damage rates in the early secondary-growth forest, biotic factors were more important in the old-growth forest, and both were associated with the proportion of damaged trees in the late secondary-growth forests.
Extreme ice storms cause severe damage to vegetation (Rhoads et al., 2002; Priebe et al., 2018; Deschênes et al., 2019). Multiple abiotic and biotic factors affect damage intensity (Bragg et al., 2003; Roženbergar et al., 2020). Our study broadly corroborates this position; however, forest-stand-specific responses differ. Late secondary-growth forests were the most vulnerable to strong ice storms, whereas early secondary-growth forests were less affected. The old-growth forests suffered only intermediate amounts. Unsurprisingly, these are generally consistent with the 'mid-domain effect' revealed by previous studies on mature forests where intermediate-sized trees sustained the most severe damage, small individuals sustained the least, and large individuals sustained an intermediate amount (Rhoads et al., 2002; Priebe et al., 2018). Early secondary-growth forests are dominated by small trees, which are prone to bending and are generally resilient when ice melts at the end of an ice storm (Bragg et al., 2003; Priebe et al., 2018). Old-growth forests are preponderantly composed of large trees and are more likely to lose canopy branches because their deep, robust root networks provide anchorage through high root-to-soil adhesion (Deschênes et al., 2019; Karban and Pearse, 2021).
According to our results, 'crown broken' was the most severely damaged type across the three forest stands, although the proportion of broken branches was higher in the old-growth forest (Fig. 3). Crown damage caused by top-breakout and branch snapping, as most of the crown losses observed in this study, may induce a high incidence of long-term mortality (Deschênes et al., 2019). Crown injuries weaken these trees because their growth and competitive viability are impaired by the loss of a photosynthetically efficient upper crown and facilitate infestation by insects and fungal pathogens, which can threaten their long-term survival (de Groot et al., 2018; Priebe et al., 2018; Karban and Pearse, 2021). Moreover, similar patterns have been reported for extreme typhoons, where crown loss is the commonest damage type and causes increased tree mortality in the long-term (Uriarte et al., 2019; Ni et al., 2021). These results underscore the necessity of conducting more surveys and monitoring studies to understand the comprehensive impact of severe glazes and other extreme climates on forests (Deschênes et al., 2019).
Our results regarding the impact of stand-specific attributes on vegetation response to severe ice storms indicate that trees that survive in the late secondary-growth forest would present more severe damage if an increase in the frequency, intensity and duration of these events occurs (Stott, 2016; Diffenbaugh, 2020; Zohner et al., 2020). These findings provide indirect evidence that the carbon cycle in late secondary-growth forests may be more sensitive to climate change than it was previously (Requena Suarez et al., 2019). Studies have shown that late secondary-growth forests tend to be rapidly increasing carbon sinks owing to high carbon sequestration rates (Requena Suarez et al., 2019; Heinrich et al., 2021). The most severe damage in late secondary-growth forests could transform trees from a live carbon pool into dead and subsequent carbon sources (Fig. 2). More importantly, regional and global scale studies have found that climatic extremes alter forest net biomass and dynamics and even weaken their carbon sinks (Curtis et al., 2018; Zohner et al., 2020). Our findings show that late secondary-growth forests suffered more damage than early secondary-growth and old-growth forests thus advancing knowledge about how forest stands respond differentially to global climate change.
Multiple biological factors related to vegetation attributes and site physical conditions are generally thought to affect the intensity of the damage caused by ice storms (Bragg et al., 2003; Deschênes et al., 2019; Klopčič et al., 2020). In the present study, our examination of the impacts of a set of abiotic and biotic factors on forest susceptibility to extreme ice storms identified the possible causes of the differences in responses across forest stands and damage types. Consistent with previous studies on temperate mature forests in North America and Europe (Bragg et al., 2003; Priebe et al., 2018; Klopčič et al., 2020), our results showed that biotic factors (i.e., forest structure and composition) play an essential role in the vulnerability of old-growth forests to ice storms. However, secondary-growth forests were not found with similarly influencing trajectories with old-growth forests (Table 1 and Table S1). Interestingly, several studies have shown that forest structure and composition have similar effects on secondary and old-growth forests to wind-related climatic extremes (e.g., hurricane, Kupfer et al., 2008; Ni et al., 2021; windstorm, Bouchard et al., 2009). These findings indicate that the factors determining damage intensity are complex and may result from interactions between the disturbance properties and multiple biotic and abiotic factors (Deschênes et al., 2019; Klopčič et al., 2020).
In line with our third prediction, topographic factors were strongly associated with the damage rates in early secondary-growth forests. The prevalence of two severe types of damage (i.e., 'clear-bole broken' and 'uprooting') with slope steepness in the early secondary-growth forest could be explained by the tendency of trees to develop crowns that are asymmetrical in a down-slope direction (Lafon, 2004; Roženbergar et al., 2020), as well as the 'domino effect' where the falling trunks topple or break the adjacent trees on steep slopes (Rhoads et al., 2002). Our study detected noticeable positives between slope aspect with clear-bole and branch breakage in the early secondary-growth forest, which contradicted previous findings in mature forests (Priebe et al., 2018; Klopčič et al., 2020) and bamboo plantations (Zhou et al., 2011), as well as our results in late secondary-growth forests (Table 1 and Table S1). Researchers generally agree that, in the Northern Hemisphere, south-facing slopes are exposed to more sunlight and receive more heat in winter than north-facing slopes, producing a warmer microclimate and consequently decreasing the duration of ice accumulation and load, which can eventually reduce the damage intensity. The habitat filtering effect toward fast-growing pioneer species in early secondary-growth forests may contribute to this inconsistency, as these early-successional species have less wood strength and their boles and branches are highly prone to breaking (Priebe et al., 2018; Song et al., 2018; Lu et al., 2020). Unexpectedly negative correlations were observed between litter thickness with uprooting and branch breakage. One possible explanation is that thick litter with high nutrient input causes tree root network development in shallow soil, which reduces root-to-soil anchorage to resist uprooting (Song et al., 2018) and facilitates the rapid growth of pioneer species that aggravate bole and branch breakage (Lu et al., 2020).
By contrast, we found that forest structure and composition had significant effects on the damage intensity of old-growth forests to the ice storms, which has been well documented in many previous studies on temperate mature forests (Deschênes et al., 2019; Roženbergar et al., 2020). Our finding that clear-bole breakage in this forest stand was positively and negatively correlated with the height of the tree layer and the basal area of the tree layer, suggests that slender-taller trees are vulnerable to mechanical instability owing to the effects of self-weight, ice accumulation or both. Meanwhile, negative relationships between clear-bole breakage with the basal area of shrub layers and shrub to tree density ratio likely reflect that denser understory shrub layers may shore up trees to resist glaze damage (Priebe et al., 2018). The slope gradient in the old-growth forest showed a similar influencing trajectory to the clear-bole breakage in the early secondary-growth forest. Crown breakage was significantly positively correlated with the height of the shrub layer, but negatively correlated with the basal area of the shrub layer and soil hardness. Thick shrubs grown in hard soils can increase root-to-soil adhesion and anchorage (Deschênes et al., 2019; Taylor et al., 2019; Karban and Pearse, 2021), which may explain this pattern. Strikingly, bulky trees with less support from denser understory shrub layers could anchor tree stems to resist glaze damage (Priebe et al., 2018), but caused an increasing trend in branch breakage.
As an intermediate stage between early secondary-growth and old-growth forests, the damage intensity of late secondary-growth forests was highly affected by both abiotic and biotic factors. Ice storm damage tended to be biased toward trees grown on north-facing slopes, likely because of colder microclimates. A denser understory shrub layer may shore up tree crowns to resist top breakouts (Rhoads et al., 2002; Deschênes et al., 2019). More interestingly, stem breakage unexpectedly negatively correlated with tree density, running counter to generally 'domino effect' trends in glaze damage (Rhoads et al., 2002). However, this was consistent with hurricane damage, in which the percentage of damaged trees was negatively dependent on stem density, probably because of the buffering effects of neighboring tree stems (Garrido-Pérez et al., 2008; Taylor et al., 2019). Thus, our findings in late secondary-growth forests suggest that high density of trees may help reduce tree damage by acting as a buffer against severe ice storms.
Irregular disturbances, such as extreme ice storms, are challenging when managing forests, and the sheer number of influencing factors makes it difficult to predict stand-level responses. Unlike most previous studies that focused on mature forests (e.g., Priebe et al., 2018; Roženbergar et al., 2020) or commercial plantations (Bragg et al., 2003; Zhou et al., 2011) in the northern temperate region, this study provides evidence regarding stand-specific differential damage from ice storms and the operating trajectories of abiotic and biotic factors to damage intensity in subtropical forests. We found that the damage to the late secondary-growth forest was the most severe, that to the old-growth forest was moderate, and the influencing factors were stand-specific across the forest stands. Although our results, obtained from only two areas, may present site-level bias, our 36 transect sites with 108 monitoring plots and statistical procedures with random effect could reduce this bias. Oak forest is a keystone and widespread vegetation type in Asia (including China). Moreover, given that extreme ice storms, as injurious disturbances, are predicted to be frequent in subtropical forest ecosystems (Gnanamoorthy et al., 2021; IPCC et al., 2021; Zohner et al., 2020; Girardin et al., 2022), these findings on stand-specific susceptibility and associated factors may have important implications for future biodiversity conservation and forest management (including reforestation) under climate change.
Given that the present study site, the Shennongjia World Natural Heritage Site, is located in a national and international biodiversity conservation hotspot (Chen et al., 2019), the key goals of managers are biodiversity and ecosystem conservation. Decisions on risk-mitigation strategies for this important region should be made cautiously and should entirely meet the above goals. With regard to management practices, we recommend that damaged trees in late secondary-growth and old-growth forests should not be removed following ice storms. With regard to management practices, although the removal of damaged trees was designed for timber production in the many post-storm restoration projects of economic forests (Haight et al., 1996), we recommend that damaged trees in late secondary-growth and old-growth forests should not be removed following ice storms (Fig. S8; Yorks and Adams, 2003; Cao et al., 2020), allowing the forest to develop heterogeneous multi-level and multi-aged structures, as gap-generating disturbances contribute to structural development. For the early secondary-growth forest, although our results suggest that slow-growing hardwood species, which can resist storms and promote long-term carbon sequestration, should be preferred for reforestation (Ni et al., 2021; Girardin et al., 2022), fast-growing, short-lived species are favored by numerous reforestation projects designed for wood productions. Short-term solutions are attractive. However, forest restoration and regeneration are long-term processes that can take centuries (Chazdon, 2008). Moreover, several other factors such as subsequent damage by insects and pathogens, summer aridity and warming winters may also affect post-storm forest restoration (Deschênes et al., 2019; Lu et al., 2020). These considerations have merit in that stress factors compound with each other, affecting tree growth and forest restoration (Chazdon, 2008; De Grandpré et al., 2019). However, extending our results to larger scales and multiple ecosystems should be done cautiously because of site- and/or species-specific responses and adaptations to climatic extremes. Therefore, long-term studies at multiple sites for diverse forest stands and types, should be the focus of future studies to provide information for comprehensive assessments of the impacts of ice storms and to inform global climate change mitigation schemes for forest conservation.
Jinyu Guo, Jifa Cui: Conceptualization, Data curation, Formal analysis, Investigation, Methodology, Visualization, Writing - original draft. Nan Wu, Jie Wang: Conceptualization, Data curation, Formal analysis. Yaqian Zhang, Hanyu Xiang, Baoshuang Hu: Investigation. Youbing Zhou: Conceptualization, Methodology, Writing - review & editing, Funding acquisition, Supervision.
All data will be provided and submitted to community-approved public repositories when this manuscript will be accepted.
The authors declare that they have no known competing financial interests or personal relationships that could have appeared to influence the work reported in this paper.
The authors would like to acknowledge Zuzhou Jin, Wanxi Zou, Zhenfei Zheng, Juncheng Li, Yao Wang, Yaoxin Qin, Qian Qian, Liyu Li, Tian Tian, Yaoyao Tian, Jian Liu, Qian Wang, Mengqing Fu, Mingjiang Zou, Yimei Yan and Dahu Zou for their assistance with fieldwork. We are grateful to Junwei Liu for early discussion about research design. We acknowledge the Administration Bureau of the Shennongjia World Natural Heritage Site for granting us permission to conduct this study.
Supplementary data to this article can be found online at https://doi.org/10.1016/j.fecs.2023.100119.
Factor | Early second-growth forest | Late second-growth forest | Old-growth forest | ||||||||||||||||
Category | Parameter | UP | CBB | CB | BB | TO | UP | CBB | CB | BB | TO | UP | CBB | CB | BB | TO | |||
Topography | Slope gradient | ↑*** | ↑*** | ↑*** | – | – | – | – | – | ↑*** | |||||||||
Slope aspect | ↑* | ↑** | ↑*** | ↓**** | ↓** | ↓** | ↓**** | – | – | – | – | – | |||||||
Soil | Soil hardness | ↓* | ↓** | ↓** | |||||||||||||||
Percent of bare rock | |||||||||||||||||||
Forest structure | Height of tree layer | ↑** | ↑*** | ↑* | ↑** | ↑*** | |||||||||||||
Basal area of Tree layer | – | – | – | – | – | – | – | – | – | – | ↓** | ↑* | |||||||
Height of shrub layer | – | – | – | – | – | ↑** | ↑*** | ↑*** | |||||||||||
Basal area of shrub layer | – | – | – | – | – | ↓* | ↓** | ↓** | ↓** | ||||||||||
Forest composition | Density of tree layer | – | – | – | – | – | ↓** | ↓*** | ↓**** | – | – | – | – | – | |||||
Density of shrub layer | – | – | – | – | – | – | – | – | – | – | |||||||||
Height ratio of shrub to tree | – | – | – | – | – | – | – | – | – | – | – | – | – | – | – | ||||
Basal area ratio of shrub to tree | – | – | – | – | – | – | – | – | – | – | – | – | – | – | – | ||||
Density ratio of shrub to tree | – | – | – | – | – | – | – | – | – | – | ↓* | ↓* | ↓** | ||||||
Nutrient input | Litter thickness | ↓** | ↓* | ↓*** | ↑* | ||||||||||||||
Note: "↑" indicates a positive coefficient, and "↓" indicates a negative coefficient. "‒" indicates a variable that was not included in the final most plausible model. Significance levels of the explanatory variables (see Table S3 for statistical values) are shown as: *, P < 0.1; **, P < 0.05; ***, P < 0.01; ****, P < 0.001. |